To understand the motivation behind the definition of the family of operators \(\{ U_{\theta}(s)\}\) by the initial-value problem of the first-order differential Eq.(2.B.2) with initial condition Eq.(2.B.3), one should contextualize what Weinberg is trying to achieve in App.B of Ch.2 (Vol.I). If one returns to Section 2.7, on "Projective Representations," one reads that Weinberg is interested in proving a "key theorem that governs the occurrence of intrinsically projective representations," namely, that the phases of the composition rule,
\[U[T(\theta_1)]U[T(\theta_2)]=e^{i\phi(\theta_1,\theta_2)} U[T(\theta_1)T(\theta_2)],\]
may be chosen such that \(\phi = 0\) whenever: (i) the generators \(\mathfrak{t_a}\) of the representation can be defined in such a way as to eliminate the central charges, i.e., \([\mathfrak{t}_a,\mathfrak{t}_b]=iC^c_{\, ab} \mathfrak{t}_c\); and (ii) the group manifold \(G\) is simply-connected.
Remark. Such a result finds an analogue in the mathematical literature in a theorem due to V. Bargmann, published in his paper "On Unitary Ray Representations of Continuous Groups" (1954), (PDF via JSTOR). A simplified proof of Bargmann's theorem was later given by D. J. Simms (1968), see Ch.2 of his monograph "Lie Groups and Quantum Mechanics" (Springer-link). In a modern mathematical notation, Bargmann's theorem may be re-formulated as follows:
-- Let \(G\) be a connected and simply-connected finite-dimensional Lie group with trivial second cohomology class, i.e., \(H^2(\mathfrak{g},\mathbb{R})=0\). Moreover, let \(\mathcal{H}\) be a separable complex Hilbert space, \(\mathbb{P}(\mathcal{H})\) its projectivization with quotient mapping \(\varphi:(\mathcal{H}-\{0\})\longrightarrow\mathbb{P}(\mathcal{H})\) , denote by \(U(\mathcal{H})\) the unitary group of \(\mathcal{H}\), define the mapping \(\hat{\varphi} :U(\mathcal{H}) \longrightarrow \mathrm{Aut} (\mathbb{P}(\mathcal{H}))\) by letting \(\hat{\varphi}(U)(\hat{x}) = \varphi(U(x)) \) whenever \(\hat{x}=\varphi(x), x\in(\mathcal{H}-\{0\})\), for all \(U \in U(\mathcal{H})\); and let \(P(\mathcal{H})=\hat\varphi(U(\mathcal{H}))\) be the subgroup of \(\mathrm{Aut} (\mathbb{P}(\mathcal{H}))\) of unitary projective transformations. Then Bargmann's theorem asserts that every projective transformation \(P \in P(\mathcal{H})\) can be lifted to an unitary transformation \(U \in U(\mathcal{H})\) such that: \(P=\hat\varphi \circ U\). If homological algebra is to your taste, you can find a proof of this theorem using Montgomory-Zippin in Schottenloher (2008), Ch.4 (PDF via author's homepage).
As a first step to motivate Eq.(2.B.2), it is helpful to show how Weinberg's notation is related to the more conventional notation in Lie theory. Let \(G\) be a simply-connected n-dimensional analytic Lie group. As such, we can adopt one of the canonical coordinates \(\theta=\{\theta^a\}_{1\leq a \leq n}\) on \(G\) . Recall that if \(\mathfrak{g}\) is solvable, the canonical coordinates of the second kind (also known as Malcev's coordinates) yields a diffeomorphism \(\theta:G\longrightarrow \mathbb{R}^n\). Therefore, we assume \(\mathfrak{g}\) is solvable only in order to avoid worrying about open neighborhoods, and we denote by \(T=\theta^{-1}\) its inverse from \(\mathbb{R}^n\) into \(G\). To obtain Weinberg's notation, i.e., Eq.(2.2.15), introduce the "multiplication map" by:
\[f^a(\theta_1,\theta_2)=\theta^a(T(\theta_1)T(\theta_2)), \,\,\, \forall \theta_1,\theta_2\in \mathbb{R}^n.\]
It follows that the mappings \(f^a\) belongs to \(C^\omega(\mathbb{R}^n\times\mathbb{R}^n;\mathbb{R})\), and the group structure (namely, the existence of the identity, which is mapped to the origin by the Malcev coordinates, \(\theta(e)=0\), and the associativity of multiplication) implies:
\[f^a(\theta,0)=f^a(0,\theta)=\theta, \,\,\, \mathrm{(I)} \\ f^a(f(\theta_1,\theta_2),\theta_3)=f^a(\theta_1,f(\theta_2,\theta_3)), \,\,\, \mathrm{(II)}\]
for all the \(\theta_j \in \mathbb{R}^n\). Using the analytic condition with the group identity, it is easy to verify that the structure of the power series expansion of each \(f^a\) is very constrained, e.g., one finds
\[f^a(\theta_1,\theta_2)=\theta_1^a+\theta_2^a+f^a_{\, bc} \theta_1^b \theta_2^c+\mathcal{O}(3), \,\,\, \mathrm{(III)}\]
where \(C^a_{\, bc}=-f^a_{\, bc}+f^a_{\, cb}\) are the structure constants. Moreover, recalling that:
\[h^a_{\, b}(\theta) = \Bigg[ \frac{\partial f^a(\theta_1,\theta)}{\partial \theta_1^b} \Bigg|_{\theta_1=0} \Bigg]^{-1}, \,\,\, \forall \theta \in \mathbb{R}^n\]
and applying the operator \(\partial / \partial \theta_1^c |_{\theta_1=0}\) to Eq.(II) (the associativity condition), one obtains:
\[\frac{\partial f^a(\theta_2,\theta_3)}{\partial \theta_2^b} h^c_{\, a}(f(\theta_2,\theta_3)) = h^c_{\,b}(\theta_2), \,\,\, \mathrm{(IV)}\]
which agrees with Eq.(2.B.7) of Weinberg (and which will be used in the first part of Weinberg's argument in App.B). Similarly, applying the operator \(\partial / \partial \theta_2^d |_{\theta_2=0}\) to Eq.(IV) and using the expansion Eq.(III) to second-order, one derives:
\[h^a_{\,b;c}(\theta) := \frac{\partial h^a_{\,b}(\theta)}{\partial \theta^c} = -f^a_{\,de} h^d_{\,b}(\theta) h^e_{\,c}(\theta), \,\,\, (V)\]
yielding Eq.(2.B.10) of Weinberg (and which is employed in the second part of App.B's argument).
After "mapping" Weinberg dialect to standard mathematical language, and deriving some useful identities to understand the argument of Ch.2, App.B, we can now motivate the first-order differential Eq.(2.B.2) -- used to later on define the family of operators \(\{ U_{\theta}(s)\}\) by using the existence-uniqueness theorem of ODE theory. Since G is simply-connected, we may assume the existence of a family of paths \(g\in G \mapsto \alpha_g \in C^1([0,1];G)\) such that \(\alpha_g(0)=e\) and \(\alpha_g(1)=g\). To obtain the notation used by Weinberg, let the family of paths in "parameter space,"
\[\theta\in\mathbb{R}^n\mapsto \Theta_\theta \in C^1([0,1];\mathbb{R}^n),\]
be defined by:
\[\Theta^a_\theta(s) := \theta^a(\alpha_{T(\theta)}(s)), \,\,\, \forall \, \theta\in\mathbb{R}^n, s\in [0,1].\]
Let \(U:G\longrightarrow U(\mathcal{H})\) be a strongly continuous unitary representation of G into a separable complex Hilbert space, and define the family of operators
\[\theta\in\mathbb{R}^n \mapsto U_\theta(s) := U[T(\Theta_\theta(s))], \,\,\, s\in[0,1].\]
Now we wish to find the differential equation satisfied by this family. Indeed,
\[\frac{d}{ds} U_\theta(s) = \frac{\partial U[T(\Theta_\theta(s))]}{\partial \theta^b} \frac{d\Theta^b_\theta}{ds}. \,\,\, \mathrm{(VI)}\]
However, the composition rule for the representation in "parameter space" reads:
\[U[T(\theta_1)]U[T(\theta_2)]=U[T(f(\theta_1,\theta_2)]. \,\,\, \mathrm{(VII)}\]
So, applying the operator \(\partial / \partial \theta^a_1 |_{\theta_1=0}\) to Eq.(VII) and using \(i\mathfrak{t}_a=\partial U[T(\theta)] / \partial \theta^a |_{\theta=0}\) (which follows from Weinberg's Eq.(2.2.17)),
\[i\mathfrak{t}_a U[T(\theta_2)]=\frac{\partial U[T(\theta)]}{\partial \theta^b} \Big|_{\theta=\theta_2} \frac{\partial f^b(\theta_1,\theta_2)}{\partial \theta_1^a}\Big|_{\theta_1=0} = \frac{\partial U[T(\theta_2)]}{\partial \theta_2^b} [h^b_{\, a}]^{-1}(\theta_2),\]
which implies:
\[\frac{\partial U[T(\theta)]}{\partial \theta^b} = i\mathfrak{t}_a U[T(\theta)]h^a_{\, b}(\theta). \,\,\, \mathrm{(VIII)}\]
Finally, using Eq.(VIII) in Eq.(VI), one arrives at:
\[\frac{d}{ds} U_\theta(s) = i\mathfrak{t}_a U_\theta(s) h^a_{\, b}(\Theta_\theta(s)) \frac{d\Theta^b_\theta}{ds}. \,\,\, \mathrm{(IX)}\]
Eq.(IX) is the differential equation whose motivation the author of this question was looking for. To close this answer, I will delineate how the argument of App.B proceeds, since we have already all the identities needed to follow it. The argument consists of two parts:
- Let \(\theta_1,\theta_2\in\mathbb{R}^n\) be the coordinates of the group elements \(g_1=T(\theta_1)\) and \(g_2=T(\theta_2)\) in G, and define the path \(\mathcal{P}\in C^1([0,1];G)\) which, in "parameter space," \(\mathcal{P}^a(s) := \theta^a(\mathcal{P}(s))\), is defined by \(\mathcal{P}^a(s)=\Theta^a_{\theta_1}(2s)\) for all \(0 \leq s \leq 1/2\) and \(\mathcal{P}^a(s)=f^a(\Theta_{\theta_2}(2s-1),\theta_1)\) for all \(1/2 \leq s \leq 1\). So notice that \(\mathcal{P}(1/2)=g_1\) and \(\mathcal{P}(1)=g_2 g_1\). Now, define the family of operators \(\{ U_{\mathcal{P}}(s) \}\) as the solution to the initial-value problem: \[\frac{d}{ds} U_{\mathcal{P}}(s) = i\mathfrak{t}_a U_{\mathcal{P}}(s) h^a_{\, b}(\mathcal{P}(s)) \frac{d\mathcal{P}^b}{ds}, \,\,\, U_{\mathcal{P}}(0)=1. \, \mathrm{(X)}\] Using Eq.(IV) derived above and the uniqueness part of the ODE theory in steps (from the identity to \(g_1\), and then from \(g_1\) to \(g_2 g_1\)), one should be able to show that: \[U_{\mathcal{P}}(1)=U_{\theta_2}(1)U_{\theta_1}(1).\]
- Computing the variation (using Eq.(IX)) \(\delta U\) with respect to \(\delta \Theta\), one shows with the help of our Eq.(V) and Weinberg's Eq.(2.B.9), that the value of \(U_{\mathcal{P}}(1)\) is the same for all the paths connecting the identity to \(f(\theta_2,\theta_1)\), so that: \[U_{\mathcal{P}}(1)=U_{f(\theta_2,\theta_1)}(1).\] Hence, \(U_{f(\theta_2,\theta_1)}(1)=U_{\theta_2}(1)U_{\theta_1}(1)\).
Remark. While it is a trivial exercise to show that if \(\rho:G\longrightarrow H\) is a Lie group homomorphism, then \(d\rho: \mathfrak{g} \longrightarrow \mathfrak{h}\) is a Lie algebra homomorphism, one should be careful when dealing with a strongly continuous unitary representation \(U:G\longrightarrow U(\mathcal{H})\). To connect the above formalism with functional analysis, one may use a theorem due to I. E. Segal (1951), in "A class of operator algebras which are determined by groups" (PDF via Project Euclid), particularly Theorem 3.1. See also Definition 3.1 to understand how to construct the "Lie algebra" of operators.
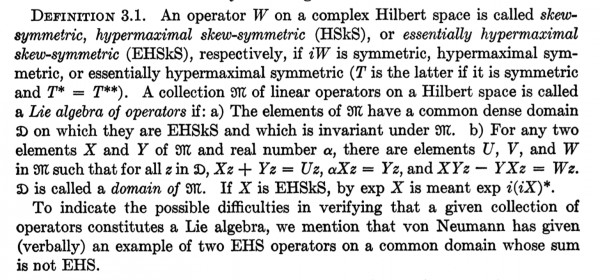
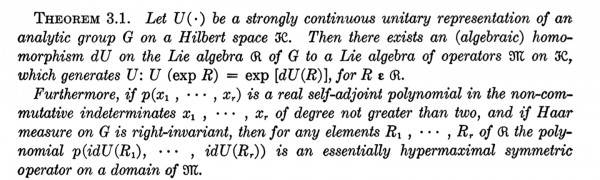