It is quite true that in GR Lie derivatives plays a fundamental role in conservation laws, but there are two different contexts elucidated in the above question. One is the conservation of quantities transported along geodesics in GR, and the other is the derivation of conserved currents in Lagrangian field theories. These concepts are different but interconnected by the idea of Killing vector fields.
Observe first that the Lie derivative depends only on a (say smooth) vector field \(\xi \in \mathcal X(M)\) on a manifold to be defined, or equivalently, its induced flow \((\phi_t)\) (1-p. group of diff such that \(\frac{d}{dt}\phi_t=\xi \circ \phi_t\)), so that the Lie derivative of a tensor \(T\in \mathrm T(M)\) is really is the rate of change along the flow lines of \(\xi\): \(\mathrm{L}_\xi T=\frac{d}{dt} \phi^*_t(T\circ \phi_t)\). (Technically, this formula is exactly true for the pull-back \(\phi_t^*\) only of covariant \(T\), which is the case of the metric discussed below. More discussion for contravariant slots, see Choquet-Bruhat and company.)
Now, the idea of a geodesic depends on another structure, a metric tensor \(\boldsymbol{g} \in \mathrm {T}^{(0,2)}M\), which also introduces the idea of parallel transport (namely, that derived from the Levi-Civita connection uniquely defined by that metric). In his book, Zee tries to make it clear that the two structures are independent:
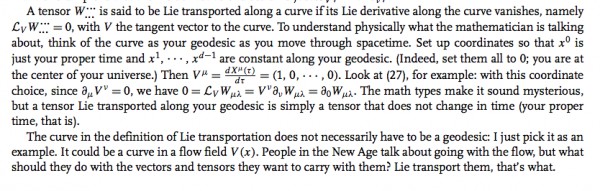
A vector field \(\xi\) is Killing iff \(\mathrm{L}_\xi \boldsymbol{g}=0\), so the rate of change of the metric along the lines of flow of that vector field vanishes, in the sense that \(\frac{d}{dt}\phi_t^*(\boldsymbol{g})=0\). This means that the family of diff generated by \(\xi\) are isometries. (Similarly, if we were interested in conformal transformations, we could "generalize" the idea of a Killing vector to only that \(\mathrm{L}_\xi \boldsymbol{g}=f\boldsymbol{g}\) for a proportionality parameter function \(f\).) These two structures, a Killing vector field and a metric, or more specifically, the family of geodesics for that metric, gives in a spacetime conserved quantities transported along the geodesic. Parametrizing the geodesic by proper-time, these quantities can be said to be conserved. In GR, this quantities are to be identified as energy, momentum, angular-momentum depending from the nature of the Killing vector. For example, if it is time-like, we associate the conserved quantity as being the energy. In the case of Lagrangian field theories, if the Lagrangian is invariant under the group of diff generated by the Killing vector, then we can construct a conserved current.
These two applications are to be discussed separately below.
General Relativity. Suppose we have a Killing \(\xi\) on a spacetime \(M\), let \(\langle X,Y \rangle = \boldsymbol{g} (X,Y)\) denote our metric and \(\nabla\) its Levi-Civita connection. Let \(\gamma:\mathbb{R}\longrightarrow M\) be a geodesic. Then the quantity \(\langle \xi\circ\gamma(s),\gamma'(s)\rangle = const.\), because
\[\frac{d}{dt} \langle \xi\circ\gamma(s),\gamma'(s)\rangle = \langle\nabla_\gamma\xi,\gamma'\rangle\]
which follows from the metric-compatibility \(\nabla \boldsymbol{g} = 0\) and the geodesic character of \(\gamma\), \(\nabla_\gamma \gamma'=0\). On the other hand, our connection being torsion less implies \(\nabla_\gamma \xi=\nabla_\xi\gamma'-\mathrm{L}_\xi\gamma'\), and we have in the last equation that:
\(\frac{d}{dt} \langle \xi\circ\gamma(s),\gamma'(s)\rangle = \frac{1}{2}\nabla_\gamma\langle \gamma',\gamma'\rangle-\frac{1}{2}[\mathrm{L}_\xi(\boldsymbol{g}(\gamma',\gamma')-(\mathrm{L}_\xi\boldsymbol{g})(\gamma',\gamma')]=0\)
which vanishes since \(\langle \gamma',\gamma' \rangle=const.\) and the Killing property \(\mathrm{L}_\xi \boldsymbol{g}=0\).
As an application, consider the 1+1 Schwarzschild spacetime (that is, ignore equatorial and polar motion, but consider only radial ones) for \(\boldsymbol{g}=-(1-2M/r)dt\otimes dt+(1-2M/r)^{-1}dr\otimes dr\). Then \(\xi = \partial_t\) is Killing, and if we parametrize a geodesic by \(\gamma'=(dt/ds) \partial_t+(dr/ds) \partial_r\), we get that the quantity
\[E=\langle\partial_t,\gamma' \rangle=-(1-2M/r)\frac{dt}{ds}\]
is conserved. We call this the energy (for a unit mass) described by our freely falling observer. If we parametrize it by proper-time, \(\langle \gamma',\gamma' \rangle=-1\), we obtain
\[(1-2M/r)(dt/ds)^2-(1-2M/r)^{-1}(dr/ds)^2=1.\]
Isolating \(dt/ds\) and replacing in the above expression for the energy, we deduce:
\[E^2=1+\big(\frac{dr}{ds} \big)^2-\frac{2M}{r}\]
Observe that in the classical (Newtonian) limit, we have \(E \approx1+\frac{1}{2}\big(\frac{dr}{ds} \big)^2-\frac{M}{r}\) (physically, the rest energy of a unit mass plus its kinetic and potential energy contributions).
Lagrangian Field Theories. Let \(\xi\) be again a Killing vector on our space, and now let \(\mathcal {L} = \mathcal {L}(\omega,d\omega)\) be a Lagrangian. Suppose that by submitting the field potential \(\omega\) to a variation along the flow lines of \(\xi\), so that
\(\bar{\delta}\omega=\frac{d}{dt} \phi_t^*(\omega)=\mathrm{L}_\xi\omega\)
our Lagrangian changes accordingly as \(\bar{\delta}\mathcal{L}=\mathrm{L}_\xi\mathcal L\). The Lagrangian functions of interest in physics involves the metric by means of the Hodge dual, canonically in the YM form \(\Omega \wedge \star \Omega\), and here lies the importance of our assumption of \(\xi\) being Killing: since its flows are isometries, it commutes with the Hodge dual (since the latter is determined only by the metric and our orientation), so \([\bar{\delta},\star]=0\). This being the case, the variation of the Lagrangian takes the form
\[\bar{\delta}\mathcal L=\big( \frac{\delta \mathcal L}{\delta \omega} + (-1)^{N-p}\frac{\delta \mathcal L}{\delta d\omega} \big)\wedge\bar{\delta}\omega-(-1)^{N-p}\frac{\delta \mathcal L}{\delta d \omega}\wedge\bar{\delta}\omega\]
where p is the degree of the potential \(\omega\). If the theory satisfy the EL eqs. in bracket and we use the identity \(\mathrm{L}_\xi = di_\xi+i_\xi d\), we obtain the conservation law:
\[d \big( i_\xi \mathcal L+(-1)^{N-p}\frac{\delta \mathcal L}{\delta d \omega} \wedge \mathrm{L}_\xi \omega\big) = 0\]
By defining the term on bracket (up to a sign and an exact differential) as the current \(\mathcal T_{\xi} \in \bigwedge^3 M\)along \(\xi\) as given by
\(\star \mathcal{T}_\xi= i_\xi \mathcal L+(-1)^{N-p}\frac{\delta \mathcal L}{\delta d \omega} \wedge \mathrm{L}_\xi \omega\)
we have \(\delta \mathcal T_\xi=0\), where the \(\delta\) without bar is the coderivative. As an example, if you take Minkowski space \(M=\mathbb{R}^{1,3}\) and the Killing vector field as one of the coordinate vectors, say, \(\xi = \partial_\mu\), and work the Lagrangian for Maxwell EM, \(\mathcal L(A,dA) = \frac{1}{2} dA\wedge \star dA\), you can easily show that the current (defined up to a sing and total diff)
\(\mathcal T_\mu=\frac{1}{2}i_\mu dA \wedge \star dA-\frac{1}{2} dA \wedge i_\mu\star dA\)
satisfy the law \(\delta \mathcal T_\mu=0\).
(In proving this, you are going to use Maxwell equation for free space \(\delta dA = \delta \boldsymbol F=0\), which is the EL eq. for our \(\mathcal L\) written above. If coupled to a current \(\mathcal J\), so that Maxwell inhomg. eq. becomes \(\delta \boldsymbol{F} = \mathcal J\), the conservation law is going to take this coupling into account, having a new term in the right hand side dictating how the EM field exchange energy-momentum with matter currents: \(\delta \mathcal T_\mu = i_\mu\boldsymbol{F} \lrcorner\mathcal J\). When written in full components for the spatial indices, this turns out to be just the Lorentz force law.)